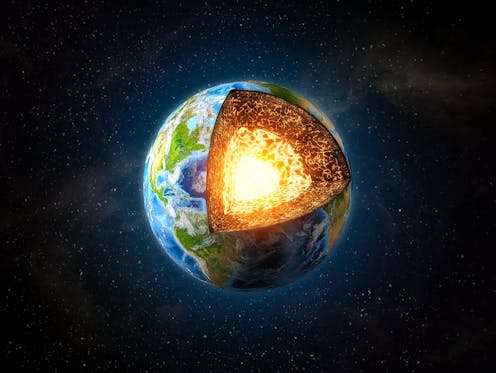
Deep beneath our feet, at a staggering depth of over 5,100km, lies Earth’s inner core — a solid ball of iron and nickel that plays a crucial role in shaping the conditions we experience on the surface. In fact, without it we’d be unlikely to even exist.
But despite its significance, it’s a bit of a puzzle how it formed and developed. We don’t even know how old it is. Luckily, mineral physics is bringing us closer to solving the mystery.
The inner core is responsible for Earth’s magnetic field, which acts like a shield, protecting us from harmful solar radiation. This magnetic field might have been important for creating the conditions that allowed life to thrive billions of years ago.
The Earth’s inner core was once liquid, but has turned solid over time. As the Earth gradually cools, the inner core expands outwards at the surrounding iron-rich liquid “freezes”. That said, it is still extremely hot, at least 5,000 Kelvin (K) (4726.85°C).
This process of freezing releases elements, such as oxygen and carbon, which aren’t compatible with being in a hot solid. It creates a hot, buoyant liquid at the bottom of the outer core. The liquid rises into the liquid outer core and mixes with it, which creates electric currents (through “dynamo action”), which generates our magnetic field.
Ever wondered what keeps the northern lights dancing in the sky? You can thank the inner core.
Cryptic crystallisation
To understand how Earth’s magnetic field has evolved over its history, geophysicists use models that simulate the thermal state of the core and mantle.
These models help us understand how heat is distributed and transferred within the Earth. They assume that the solid inner core first appeared when the liquid cooled to its melting point, taking this as the time when it began to freeze. The trouble is, that does not accurately reflect the process of freezing.
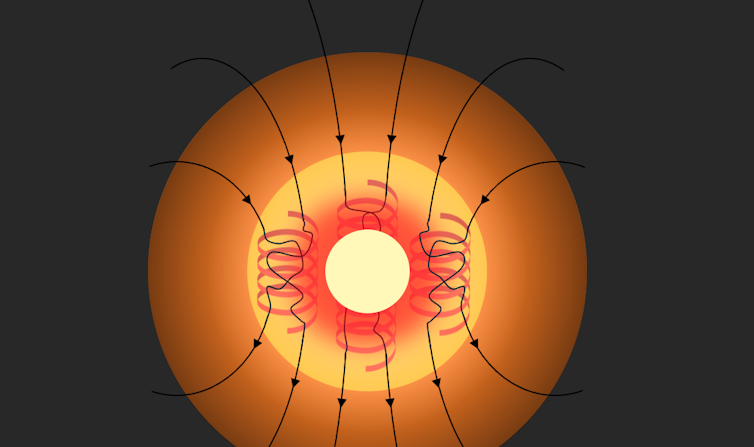
Scientists have therefore explored the process of “supercooling”. Supercooling is when a liquid is cooled below its freezing point without turning into a solid. This happens with water in the atmosphere, sometimes reaching -30°C before forming hail, and also with iron in Earth’s core.
Calculations suggest that up to 1,000K of supercooling is actually required to freeze pure iron in the Earth’s core. Given that the conductivity of the core implies it cools at a rate of 100-200K per billion years, this presents a significant challenge. This level of supercooling implies that the core would have needed to be below its melting point for the entirety of its history (1,000 to 500 million years old), which presents additional complications.
Since we cannot physically access the core — humans have only drilled 12km into the Earth — we rely almost entirely on seismology to understand our planet’s interior. The inner core was discovered in 1936, and its size (about 20% of Earth’s radius) is one of the best-constrained properties of the deep Earth. We use this information to estimate the core’s temperature, assuming that the boundary between solid and liquid represents the intersection of the melting point and core temperature.
This assumption also helps us estimate the maximum extent of supercooling that could have taken place before the inner core began to form from a combined inner and outer core. If the core froze relatively recently, the current thermal state at the inner core–outer core boundary indicates how much the combined core might have been below its melting point when the inner core first began to freeze. This suggests that, at most, the core could have been supercooled by about 400K.
This is at least double what seismology allows. If the core was supercooled by 1,000K before freezing, the inner core should be much larger than observed. Alternatively, if 1,000K is necessary for freezing and was never achieved, the inner core should not exist at all. Clearly, neither scenario is accurate, so what could be the explanation?
Mineral physicists have tested pure iron and other mixtures to determine how much supercooling is needed to initiate the formation of the inner core. While these studies have not yet provided a definitive answer, there are promising advances.
For example, we have learned that unexpected crystal structures and the presence of carbon may affect supercooling. These findings suggest that certain chemistry or structure that had previously not been considered might not require such an unreasonably large supercooling. If the core could freeze at less than 400K of supercooling, it can explain the presence of the inner core as we see it today.
The implications of not understanding the formation of the inner core are far-reaching. Previous estimates of the inner core’s age range from 500 to 1,000 million years. But these do not account for the supercooling issue. Even a modest supercooling of 100K could mean the inner core is several hundred million years younger than previously thought.
Understanding the signature of inner core formation in the paleomagnetic rock record — an archive of the Earth’s magnetic field — is crucial for those studying the impact of solar radiation on mass extinctions.
Until we better understand the magnetic field’s history, we cannot fully determine its role in the emergence of habitable conditions and life.

Alfred Wilson-Spencer receives funding from NERC grants NE/T000228/1 and NE/V010867/1.
This article was originally published on The Conversation. Read the original article.